Fluorescence Spectroscopy
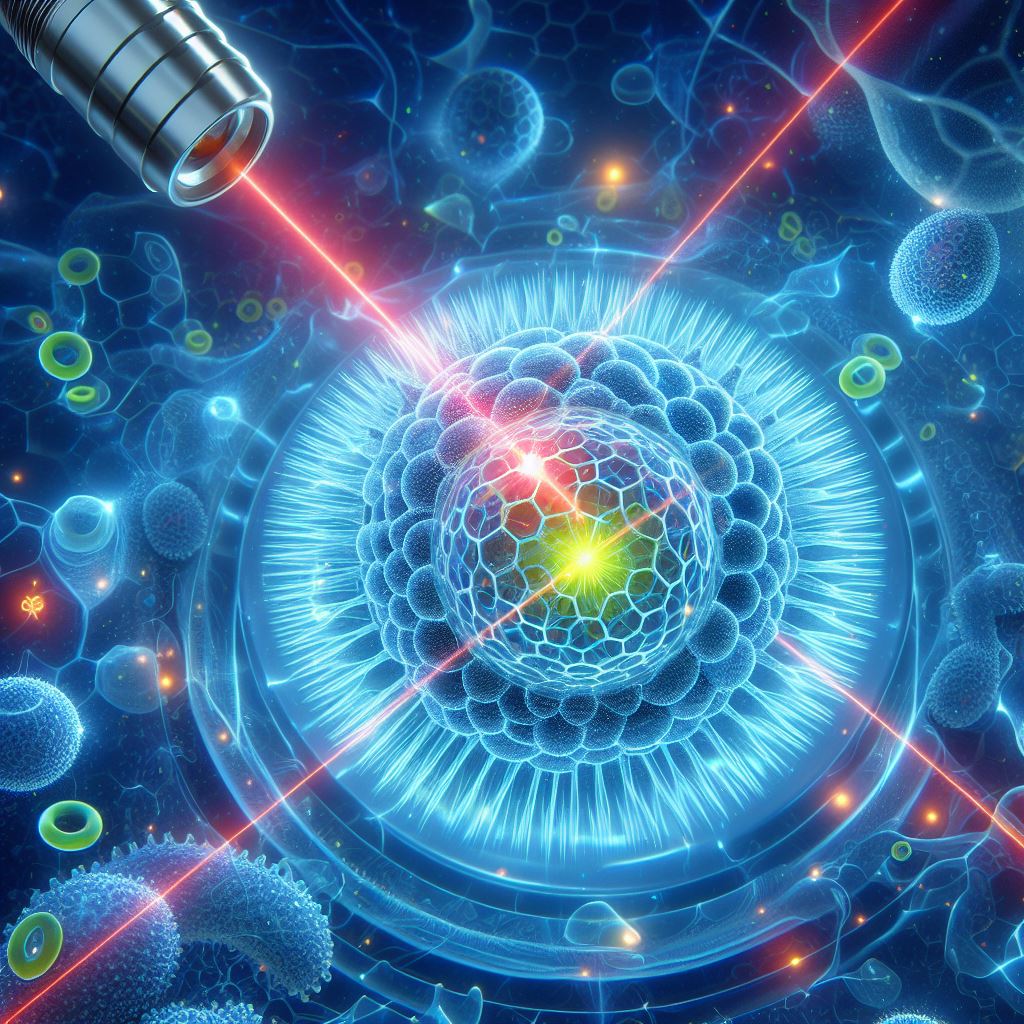
Fluorescence spectroscopy, with its origins in the early 20th century, has evolved into a sophisticated tool widely used in analytical chemistry, molecular biology, and pharmacology. The prevalence of this tool and its diverse applications can be equally attributed to developments in optics and laser technology as much as breakthroughs in the natural sciences.
What is Fluorescence:
Grounded in the principle of molecular fluorescence, this technique relies on fluorophores — compounds that are capable of absorbing and re-emitting photons at specific wavelengths. Firstly, a photon is absorbed by the fluorophore at the singlet ground electric state S0 (the lowest energy state). Within femtoseconds (10⁻¹⁵s) the excited fluorophore transitions into either the first or second singlet (S1 or S2) and one of 3 available vibrational levels (Figure 1). Within pico seconds (10⁻¹²s) it relaxes to the first vibrational level of S1 in the process of internal conversion. By doing so it loses a fraction of the energy absorbed by non-radiative decay. Lastly, the fluorophore drops down to the original state in a matter of nanoseconds (10⁻⁹ s) and by doing so emits a photon in the process known as fluorescence. The rapid nature of this process makes it particularly versatile. Consequently, techniques utilizing fluorescence, such as fluorescence activated cell sorting, are not limited by the rate of photon absorption and emission.
Figure 1. Illustration of fluorophore excitation resulting in either fluorescence or phosphorescence.
Distinction between Fluorescence and Phosphorescence:
Molecules in the S1 state can experience a spin transformation to the first triplet state, T1. Emission from the triplet state is known as phosphorescence. Because T1 and its associated vibrational levels are lower energy than S1 it is generally shifted to longer wavelengths (lower energy) compared to fluorescence. Conversion of S1 to T1 is called intersystem crossing (Figure 1.). Transition from T1 to the singlet ground state is forbidden, and as a result the rate constants for triplet emission are several orders of magnitude smaller than those for fluorescence, making it an inferior tool on its own in most applications.
Stokes Shift:
After excitation, during Internal Conversion the system transitions into a lower energy state, such as the first vibrational level of S1. The net energy available is then lower than that of the absorbed photon. In these instances the emitted light will always be of lower energy compared to the initial excited state. Because the energy of a photon is inversely proportional to its wavelength this phenomenon explains why the emitted photons are usually red-shifted (of longer wavelength). This phenomena of spectral differences between the absorbed and emitted light is known as the Stokes Shift (Figure 2). The gap between absorption and emission peaks is crucial in enabling photodetectors to discriminate between incident light used to excite the sample and signal obtained from fluorescence.
Figure 2. Absorption and fluorescence emission spectra of quinine illustrating the Stokes Shift phenomenon.
Because of the unique molecular arrangement, each fluorophore exhibits distinctive affinity for a particular wavelength of light. This can be seen in Figure 2. where the excitation for Quinine peaks at 350nm. Consequently, the emitted fluorescence serves as a unique fingerprint, providing vital information about the fluorophore's environment, concentration, and interactions. Because of these characteristics information, such as molecular conformation, binding kinetics, and cellular dynamics can be acquired with high sensitivity and specificity.
Laser Modulation in Fluorescence Decay:
Techniques such as phase-modulated fluorometry rely on fluorescence decay measurements to obtain necessary time-resolved information. The fluorophore’s lifetime, otherwise known as the excited state lifetime (τ) describes how long it will take for the the number of excited molecules to decay to 1/e (or 36.8%) of the excited state population. It is unique to a given molecule in a given environment. Fluorescence decay and excited state lifetime are closely related concepts. Fluorescence decay refers to the process by which a fluorophore returns from its excited state to the ground state, emitting a fluorescence photon in the process. While excited state lifetime is the average duration a fluorophore stays in its excited state before undergoing this fluorescence decay.
To accurately calculate the duration of fluorescence decay high precision instrumentation is necessary. In these instances laser modulation plays a crucial role, particularly in the frequency-domain or phase-modulation method. Here, the sample is excited with intensity-modulated light. The laser’s intensity is modulated at a high frequency, often near 100 MHz, forcing the fluorescent emission to respond at the same modulation frequency. Due to the fluorophore’s lifetime, the emission is delayed relative to the excitation, creating a measurable phase shift (φ). This phase shift, along with the decrease in the peak-to-peak height of the emission, can be used to calculate the decay time (Figure 3). The ability to modulate the laser’s intensity at such high frequencies allows for precise control over the excitation. This makes diode lasers particularly useful in time-resolved fluorescence measurements, enabling detailed exploration of molecular dynamics.
Figure 3. Modulated exciting light (blue) and fluorescence (red) delayed in phase (φ).
Diode Lasers in Fluorescence Spectroscopy:
In fluorescence spectroscopy, diode lasers play a crucial role in excitation, facilitating the examination of molecular structures and dynamic processes. Diode lasers are utilized as excitation sources due to their compact size, cost-effectiveness, and wavelength tunability. Their emission spectra can be tailored to match the absorption characteristics of fluorophores, ensuring optimal excitation efficiency. Diode lasers are particularly advantageous in time-resolved fluorescence studies, where their rapid on-off switching capabilities enable precise control over excitation intervals, essential for capturing fast kinetic processes. The monochromatic and coherent nature of diode laser light ensures selective and efficient excitation, contributing to improved signal-to-noise ratios in fluorescence measurements. Moreover, diode lasers find applications in various spectroscopic techniques, such as fluorescence resonance energy transfer (FRET) studies and fluorescence lifetime imaging microscopy (FLIM), enhancing the versatility and analytical capabilities of fluorescence spectroscopy across diverse scientific domains.
Most of our CW lasers are a great match for Fluorescence Spectroscopy applications, particularly in instruments requiring a compact source of monochromatic light. Our 488nm, 532nm, and 633nm wavelength lasers and wavelength combiners are focused to satisfy the needs of the fluorescence spectroscopy market. Available in both narrow and regular spectrum configurations, they can be easily integrated into systems that require a compact and precise light source.
Relevant Products
405 nm SLM Laser
Spectral line width FWHM, MHz: 20
Output power, mW: 50
Power stability, % (RMS, 8 hrs): 0.05
Intensity noise, % (RMS, 20 Hz to 20 MHz): 0.2
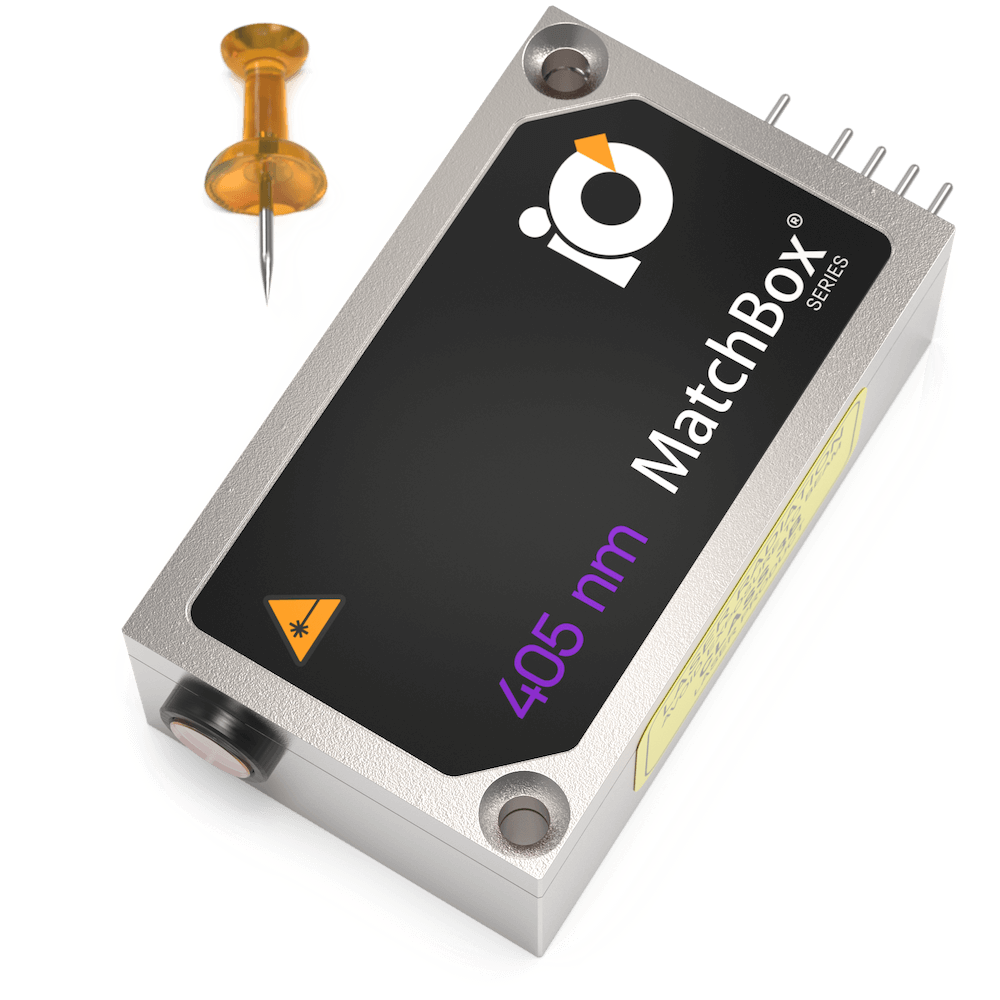
561 nm SLM Laser
Spectral line width FWHM, MHz: 2
Output power, mW: 50
Power stability, % (RMS, 8 hrs): 0.1
Intensity noise, % (RMS, 20 Hz to 20 MHz): 0.7
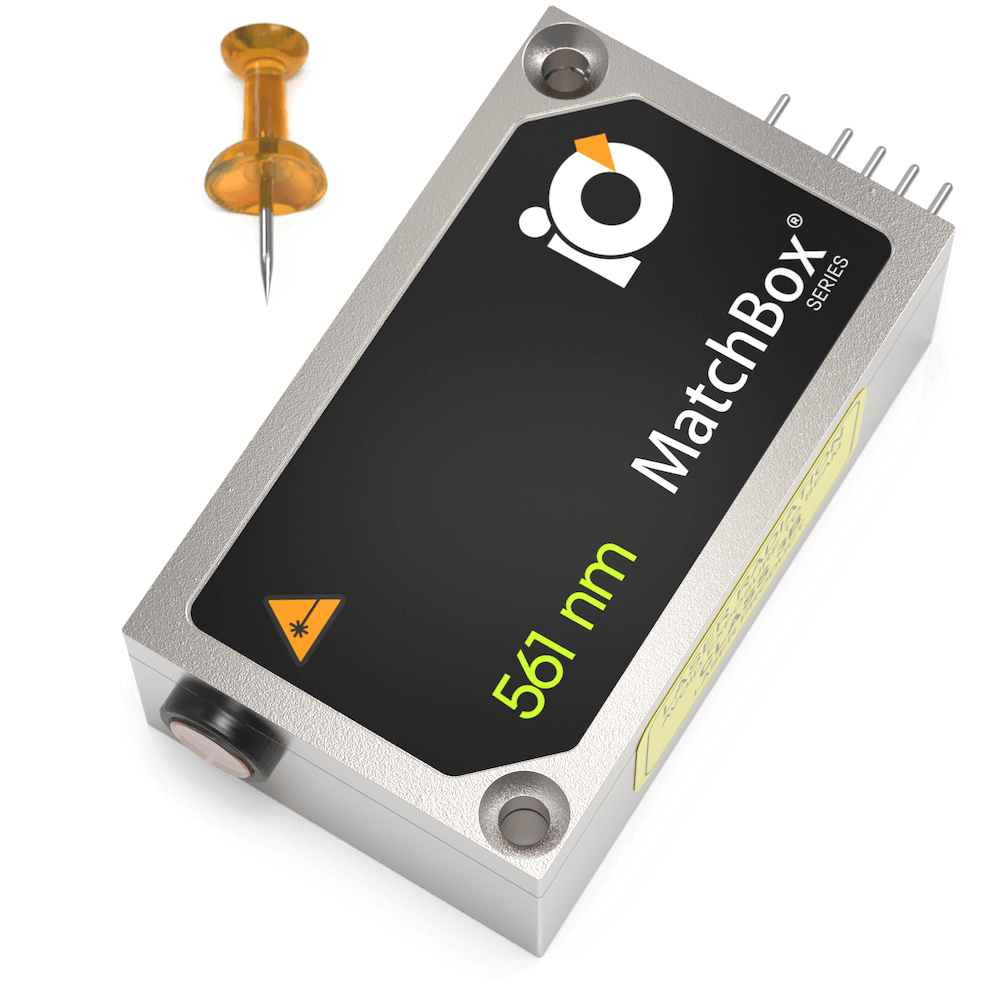
532 nm SLM Laser
Spectral line width FWHM, MHz: 2
Output power, mW: 50
Power stability, % (RMS, 8 hrs): 0.1
Intensity noise, % (RMS, 20 Hz to 20 MHz): 0.7
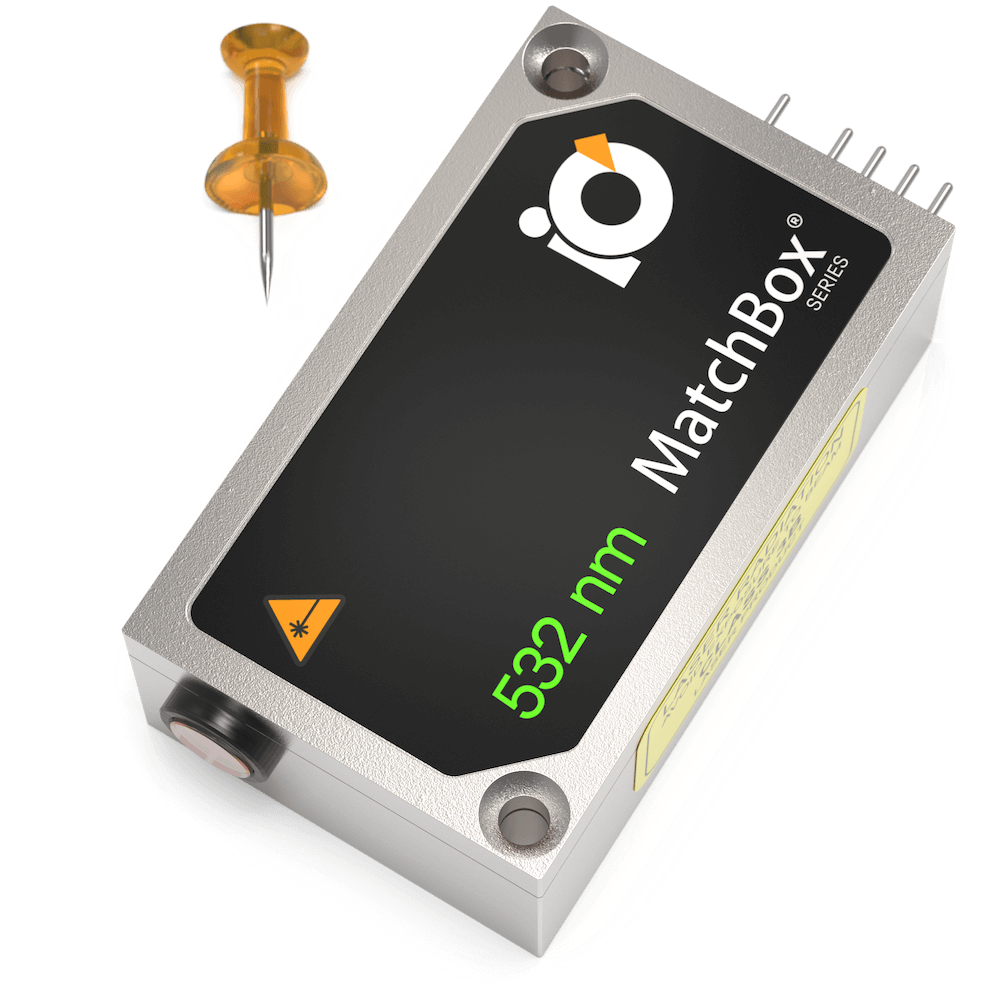
638 nm SLM Laser
Spectral line width FWHM, MHz: 2
Output power, mW: 100
Power stability, % (RMS, 8 hrs): 0.03
Intensity noise, % (RMS, 20 Hz to 20 MHz): 0.25
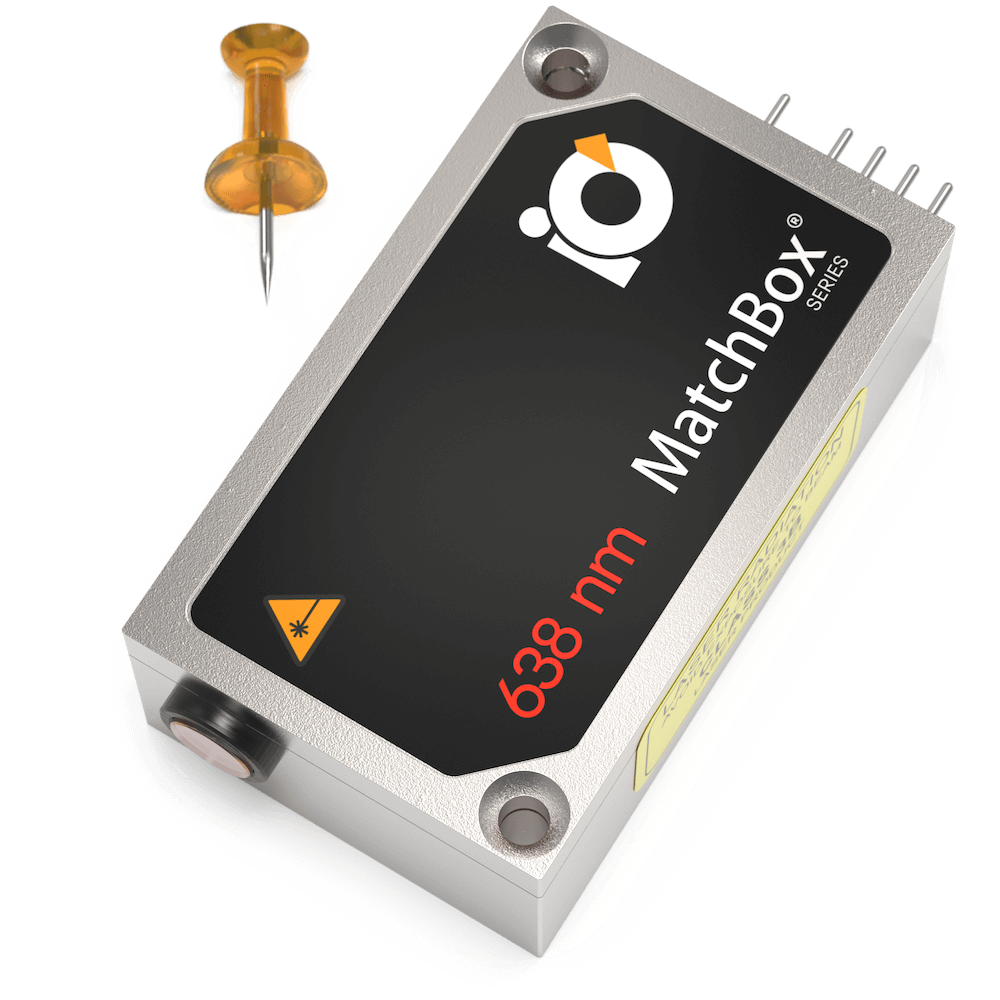
785 nm SLM Laser
Spectral line width FWHM, MHz: 2
Output power, mW: 125
Power stability, % (RMS, 8 hrs): 0.02
Intensity noise, % (RMS, 20 Hz to 20 MHz): 0.15
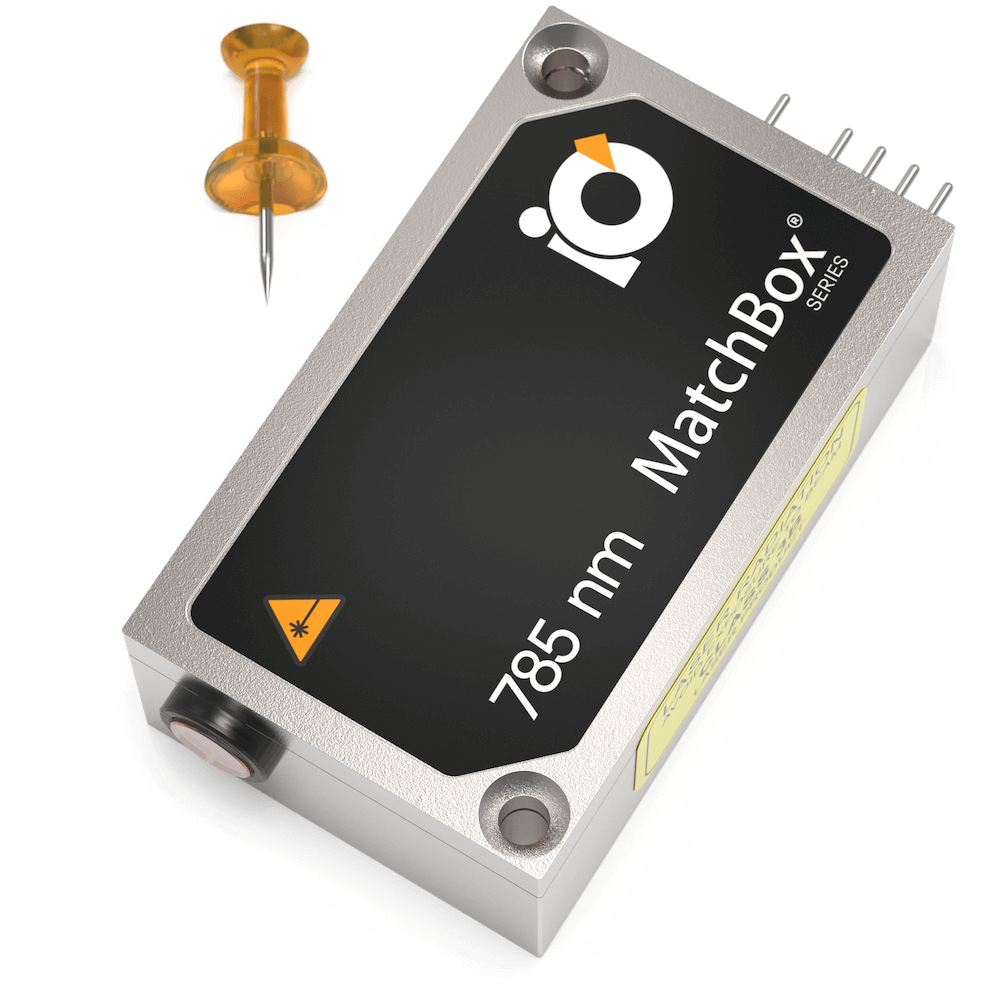
405 nm Laser
Spectral line width FWHM, nm: 0.5
Output power, mW: 180
Power stability, % (RMS, 8 hrs): 0.1
Intensity noise, % (RMS, 20 Hz to 20 MHz): 0.15
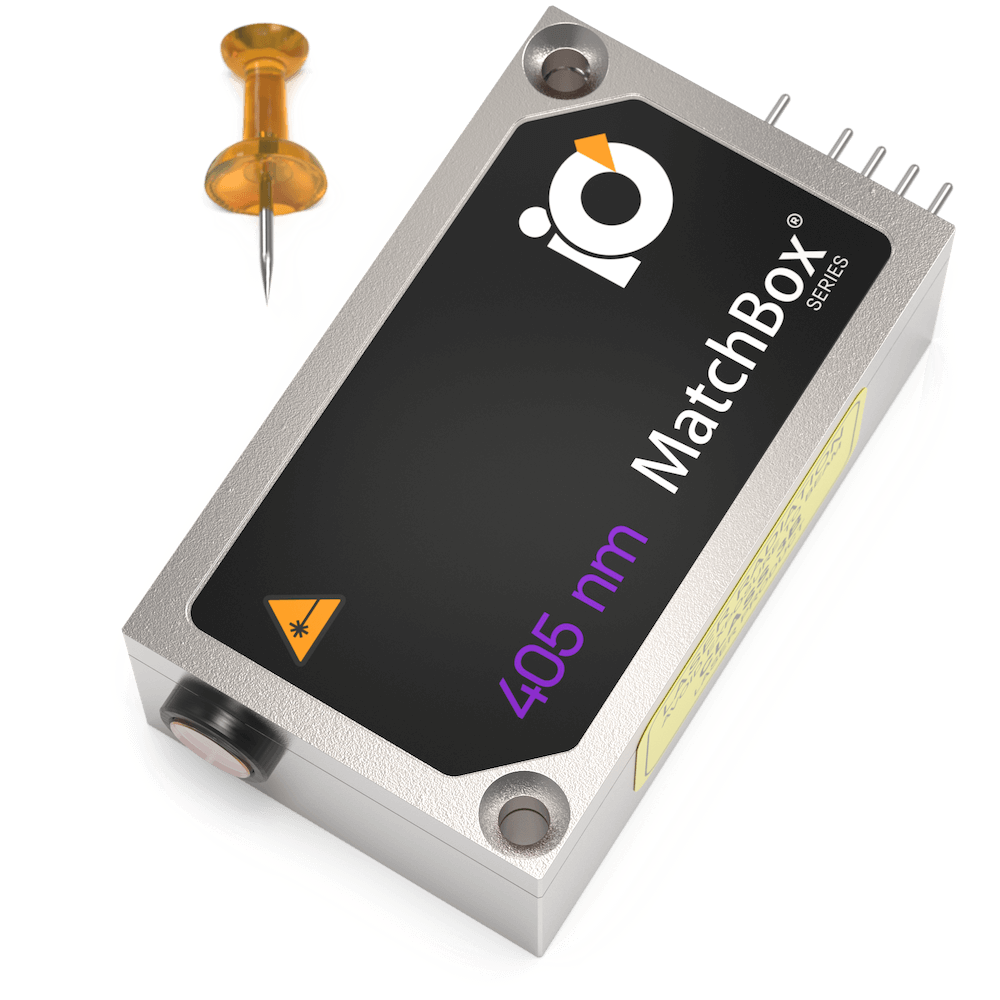
633 nm SLM Laser
Spectral line width FWHM, MHz: 2
Output power, mW: 70
Power stability, % (RMS, 8 hrs): 0.03
Intensity noise, % (RMS, 20 Hz to 20 MHz): 0.2
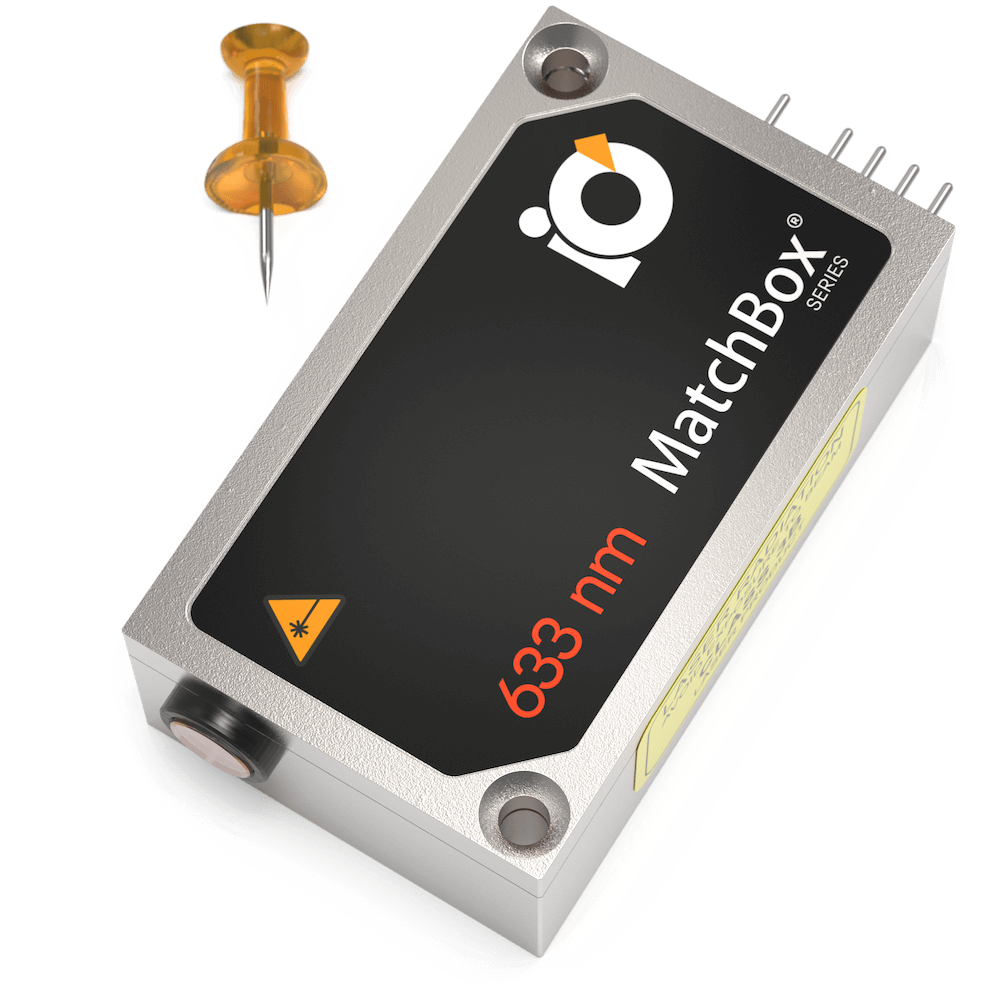
505 nm Laser
Spectral line width FWHM, nm: 0.7
Output power, mW: 60
Power stability, % (RMS, 8 hrs): 0.03
Intensity noise, % (RMS, 20 Hz to 20 MHz): 0.5
Warranty, months (op. hrs): 14 (10000)
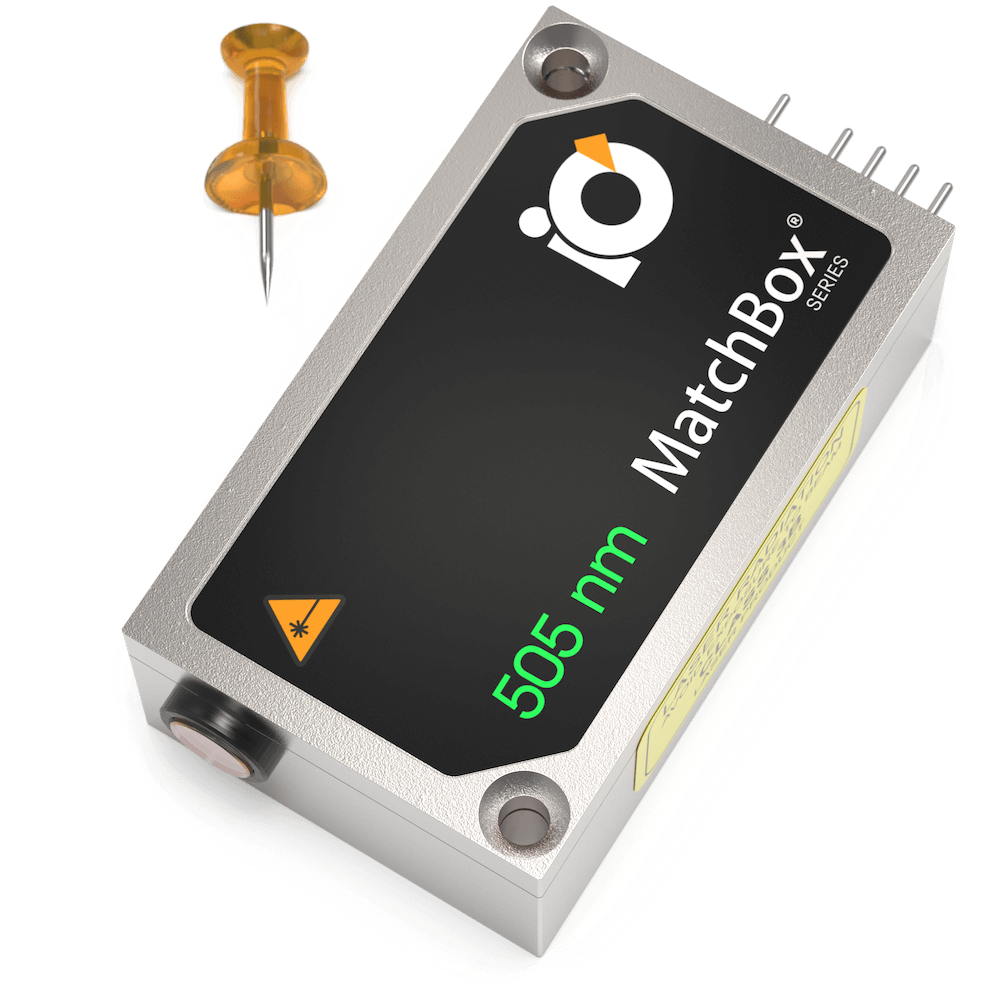
488 nm Laser
Spectral line width FWHM, nm: 0.5
Output power, mW: 40
Power stability, % (RMS, 8 hrs): 0.02
Intensity noise, % (RMS, 20 Hz to 20 MHz): 0.3
Warranty, months (op. hrs): 14 (10000)
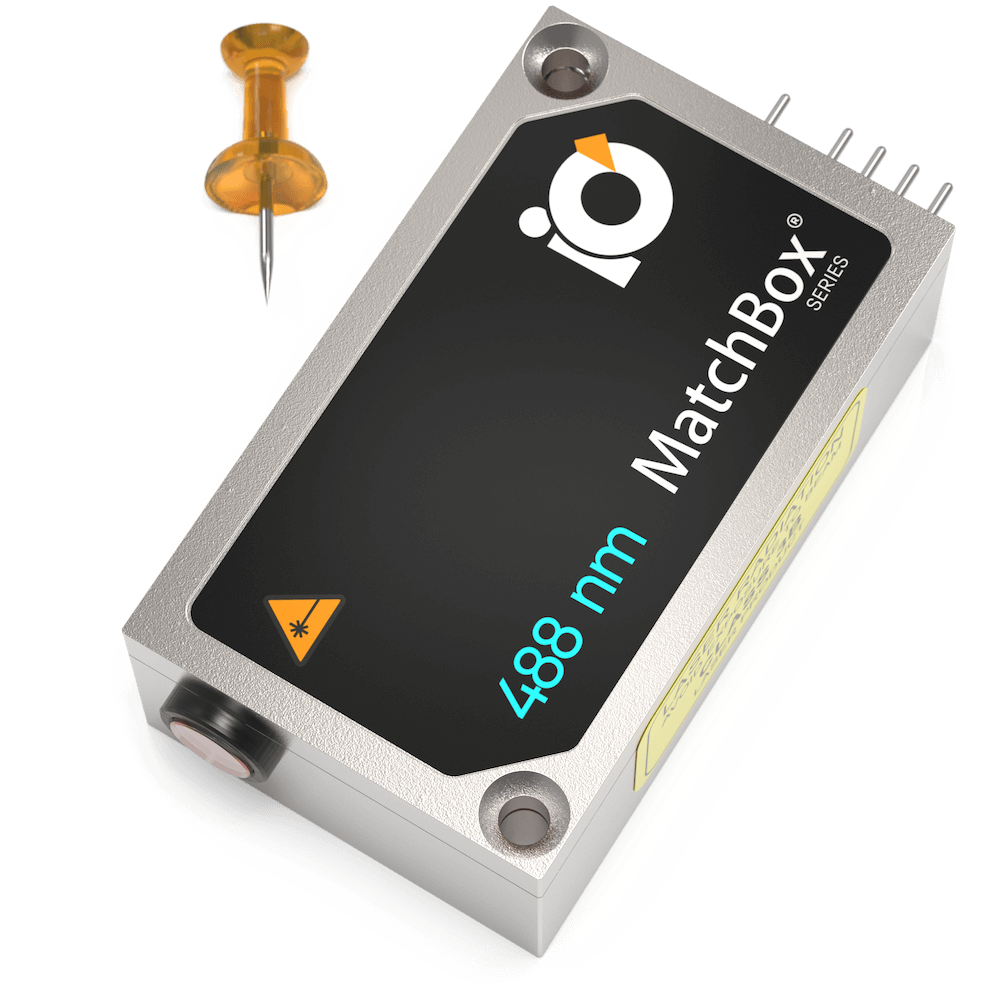
Multi-Wavelength Laser
488 nm - 40
520 nm - 80
638 nm - 130
Spectral line width FWHM, nm: 1
Power stability, % (RMS, 8 hrs): 0.2
Intensity noise, % (RMS, 20 Hz to 20 MHz): 0.5
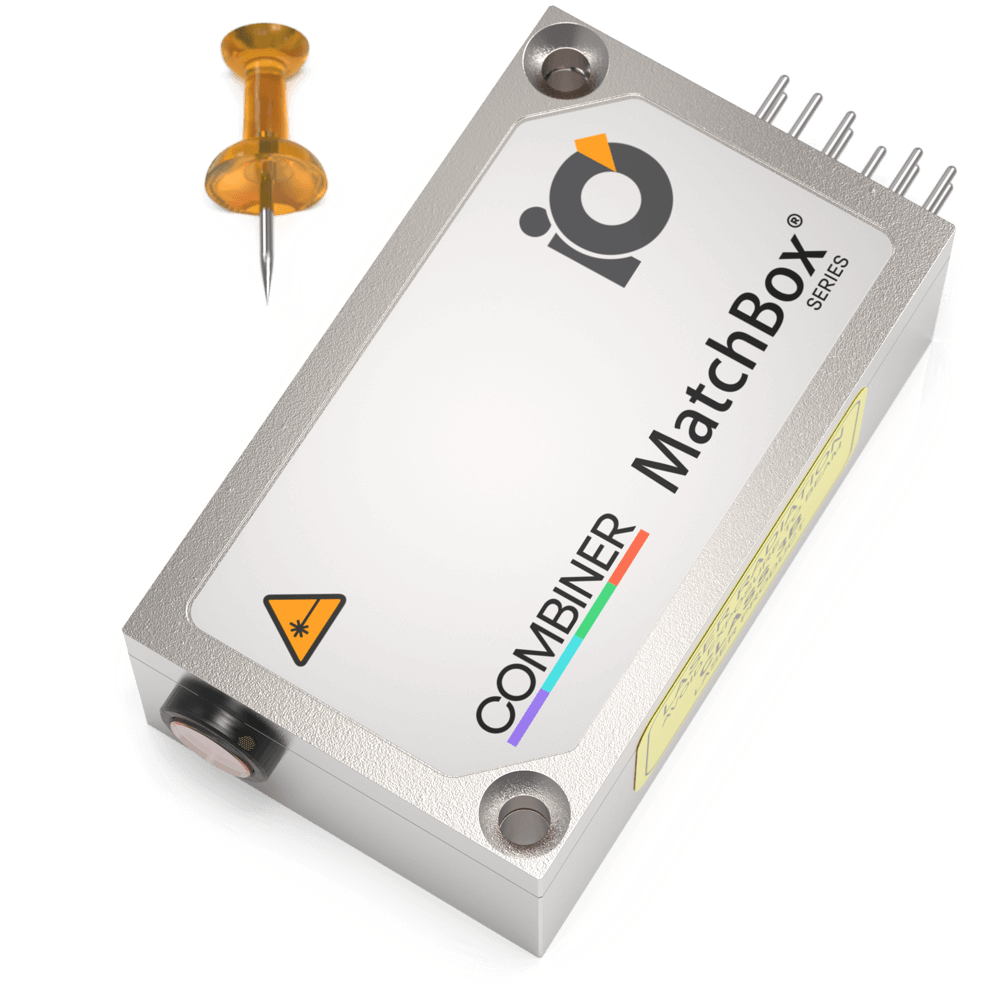