Flow Cytometry
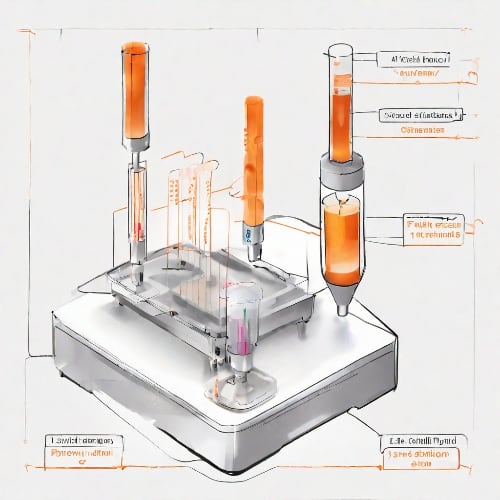
Flow cytometry is a sophisticated analytical technique widely used in biomedical research and clinical diagnostics. It allows for the simultaneous analysis of multiple physical and chemical characteristics of cells or particles as they flow through a laser beam. By utilizing fluorescence and light-scattering principles, flow cytometry provides valuable insights into cell populations, allowing researchers to study cell morphology, identify cell types, and assess various cellular functions with high-throughput precision.
Flow cytometry is a powerful technique for the analysis of multiple parameters of individual cells within a heterogeneous population. Today’s “state-of-the-art” flow cytometers are capable of measuring/detecting up to 30+ parameters per cell at rates over 10,000 cells/sec. The flow cytometer performs this analysis by passing thousands of cells per second through a laser beam and capturing the scattered light from each cell as it passes through.
Drawing the Sample
A simplified flow cytometry process begins as the sample of cells is drawn from the test tube and reaches the cytometer's nozzle. There the cell suspension is introduced into the system, and it is here that the sheath fluid is used to hydrodynamically focus the cells into a single file. The sample is streamlined through the interrogation point where the laser and the samples intersect. The suspended stream of cells passes the laser beam one cell at a time. When cells intersect the beam, light is refracted and scattered (Figure 1). With the help of dichroic/bandpass filters this light is directed to photomultiplier tubes which act as signal detectors.
Fig. 1 A simplified diagram of a Flow Cytometer using Fluorescence Activated Cell Sorting.
Detecting FSC, SSC and Fluorescence
Scattered light is registered as Forward Scatter (FSC) and Side Scatter (SSC), while any fluorescence is split into defined wavelengths and channelled by a set of filters and mirrors to separate detectors. FSC (low angle light scatter) is the amount of light scattered in the forward direction and is roughly proportional to the size of the cell. It is is measured by a detector in front of the beam. SSC (light scattering at larger angles) is caused by granularity and structural complexity inside the cell. This light is collected together with fluorescence, by separate detectors, usually located 90 degrees from the laser's path (Figure 2).
Figure 2. Optical component arrangement within the flow cytometer.
Understanding the Results
Fluorescence wavelength collected depends on the fluorophore-conjugated antibodies used. They identify cell specific markers within and on the surface of the cell. Primary antibodies attach to the markers on the cell and are not conjugated, secondary antibodies are conjugated to a fluorophore and bind to the primary antibody. This helps amplify the signal as multiple secondary antibodies can attach to one primary antibody.
These parameters (FSC, SSC, and fluorescence) provide valuable information about the cells’ size, granularity, and the presence of specific biological markers. Analog signals received by the detectors are converted into a voltage pulses. The bigger / more complex / more fluorescent the cell is, the higher voltage pulse it generates. This way the cells can be separated by different parameters and data can be collected for later analysis (Figure 3).
FFigure 3. An example of three unique populations of cells plotted along the FSC and SSC axes.
Potential of Flow Cytometry
Flow cytometry offers numerous useful features such as rapid multi-parameter analysis, cell counting and biomarker detection. However, one of the most valuable application of this technology is the ability to sort a population of heterogenous cells based on parameters of interest. The most common technique to achieve this is Fluorescence Activated Cell Sorting (FACS). This method utilizes the aforementioned fluorophore-conjugated antibodies to identify different cell populations based on their unique markers. The obtained fluorescence signal provides crucial information to determine which batch a cell will be assigned.
Principle of FACS
To successfully sort the cells after their fluorescence signal has been acquired each individual cell must first be separated from the stream and encapsulated within a single droplet. The size of the droplet will roughly correspond to the diameter of the nozzle’s opening. It is usually 40-100μm and is sufficient to contain one eukaryotic cell (living organism that is not bacteria) that is 5-100μm in diameter.
While at the integration point cells move in a single file as part of the general stream. Dropletization occurs the moment after they pass the integration point. Once the FSC, SSC and Fluorescence readings have been captured that section of the stream is partitioned into droplets encapsulating one “reading”. This is achieved by using a piezoelectric transducer which creates a sound wave along the stream. The rippling effect caused by the sound wave periodically separates the droplet ,containing the cell that was just measured, from the rest of the stream.
For the system to sort cells based on fluorescence signal it must be able to rapidly direct each droplet to the correct collection tube. This is achieved by having droplets of different charge. Charge is applied right before the droplet is formed, and is applied to the whole stream. Once the the droplet separates, the entire stream’s charge is reset to neutral and the process repeats. One at a time, the encapsulated particles reach the deflection plates. They create an electric field and depending on the charge, direct the droplets to the appropriate collection tube (Figure 1).
Diode Lasers in Flow Cytometry
Many manufacturers are opting to replace slower and bulkier solid-state lasers in flow cytometers with diode lasers, driven by several advantages. One of the reasons behind this is that more wavelengths become available for diode lasers, enabling more intricate experimental setups. At the same time, they are outcompeting the alternatives in terms of cost/performance. Moreover, Integrated Optics offers lasers whose dimensions are the smallest in the market while maintaining excellent performance. The tiny footprint of our lasers makes them a great ‘fit’ for integration into flow cytometry systems that have consistently become smaller over time. Our wavelength combiners and the model Ara were designed with flow cytometry in mind. You can switch between up to four different wavelengths including 561nm. This makes them compatible with all the most commonly used fluorescent dyes (FITC, PE, Alexa Fluora 633, SYTOX Green etc.) enabling you to cover the largest range for greatest utilization.
Relevant Products
660 nm Laser
Spectral line width FWHM, nm: 0.7
Output power, mW: 110
Power stability, % (RMS, 8 hrs): 0.05
Intensity noise, % (RMS, 20 Hz to 20 MHz): 0.2
Warranty, months (op. hrs): 14 (10000)
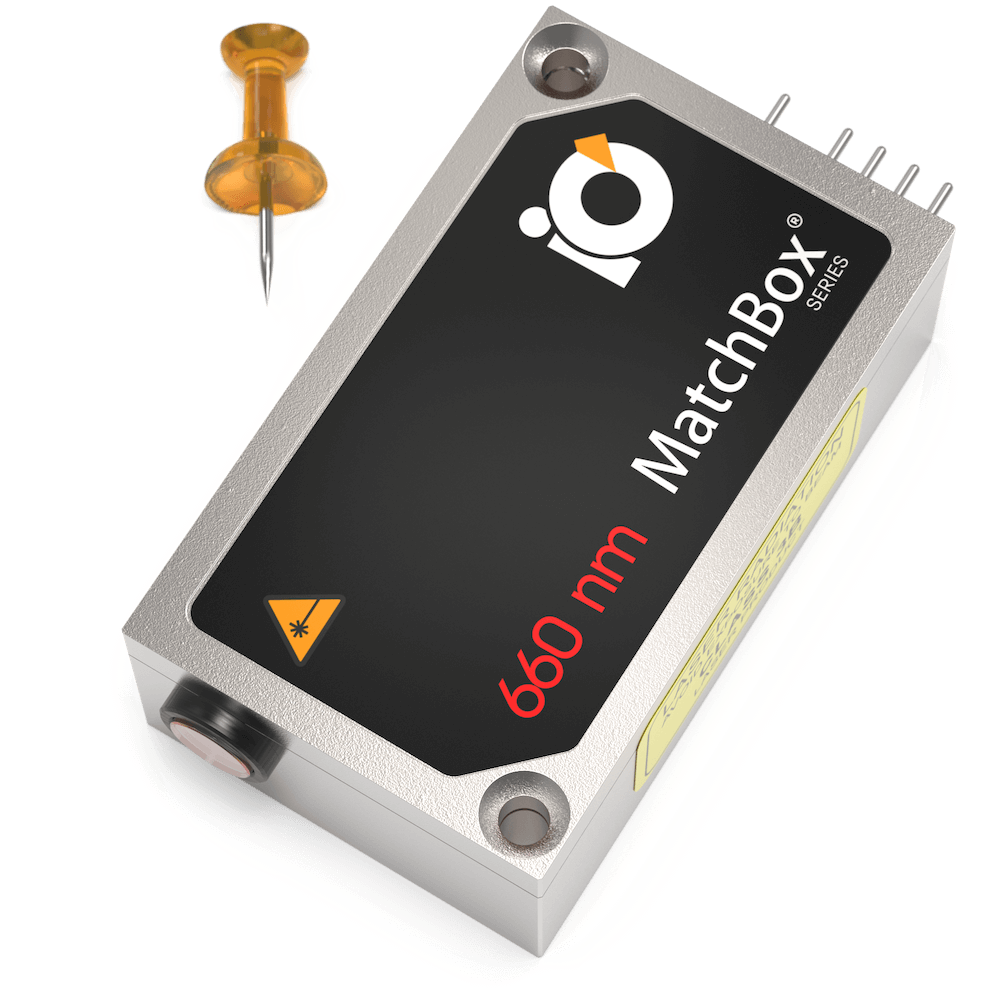
Multi-Wavelength Laser
488 nm - 40
561 nm - 50
638 nm - 130
Power stability, % (RMS, 8 hrs): 0.2
Intensity noise, % (RMS, 20 Hz to 20 MHz): 0.5
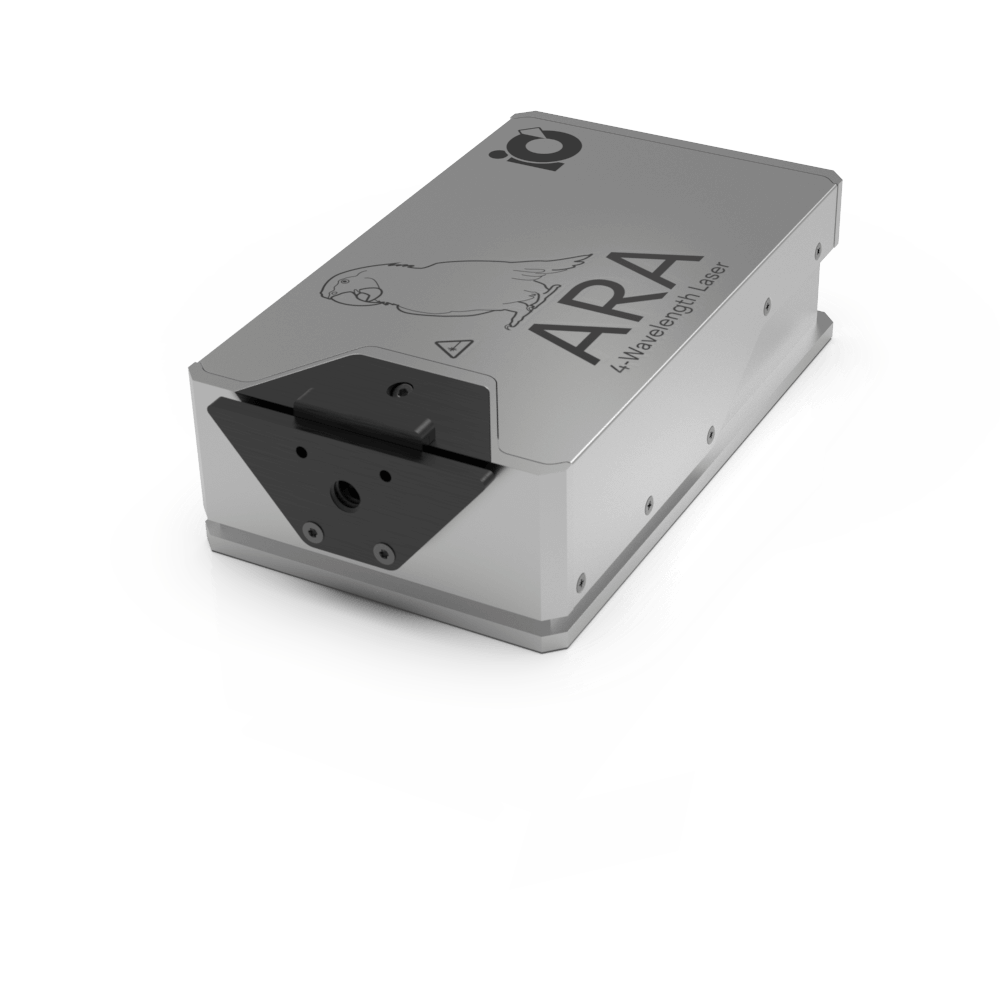
633 nm Laser
Spectral line width FWHM, nm: 0.5
Output power, mW: 90
Power stability, % (RMS, 8 hrs): 0.05
Intensity noise, % (RMS, 20 Hz to 20 MHz): 0.25
Warranty, months (op. hrs): 14 (10000)
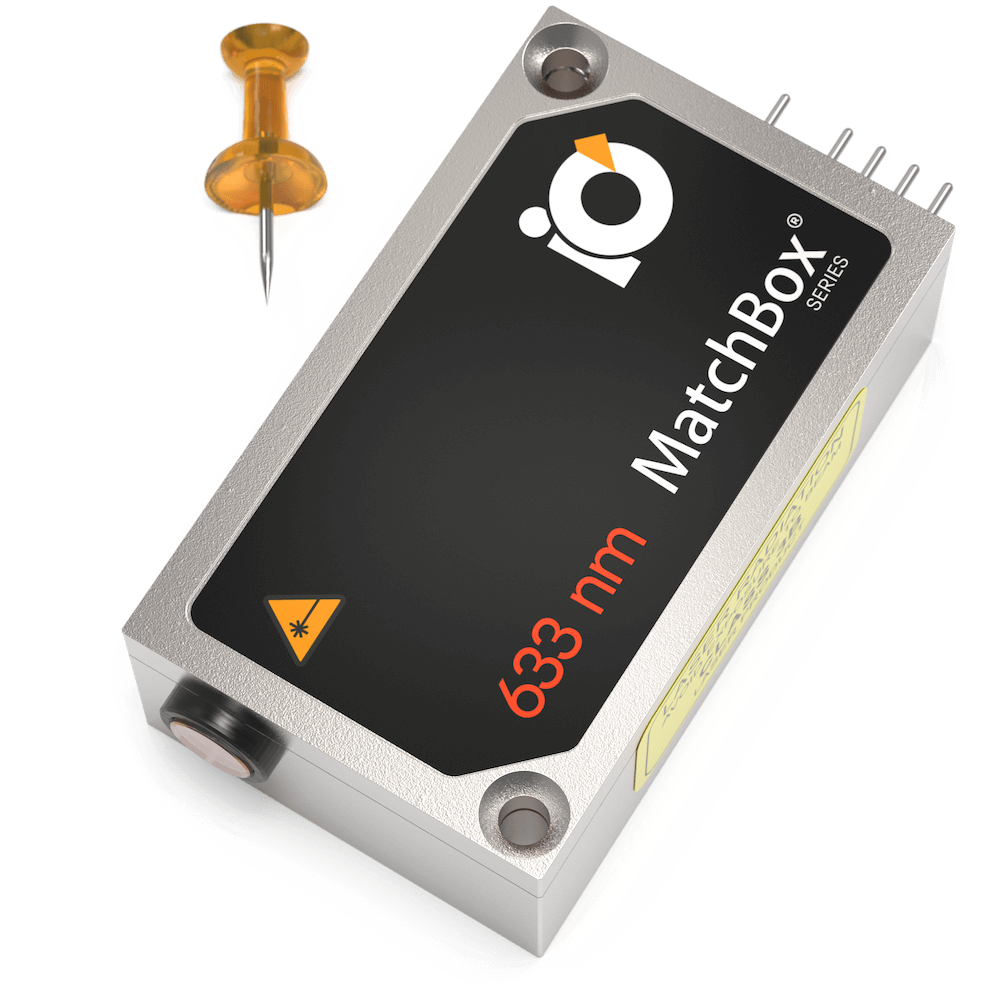
505 nm Laser
Spectral line width FWHM, nm: 0.7
Output power, mW: 60
Power stability, % (RMS, 8 hrs): 0.03
Intensity noise, % (RMS, 20 Hz to 20 MHz): 0.5
Warranty, months (op. hrs): 14 (10000)
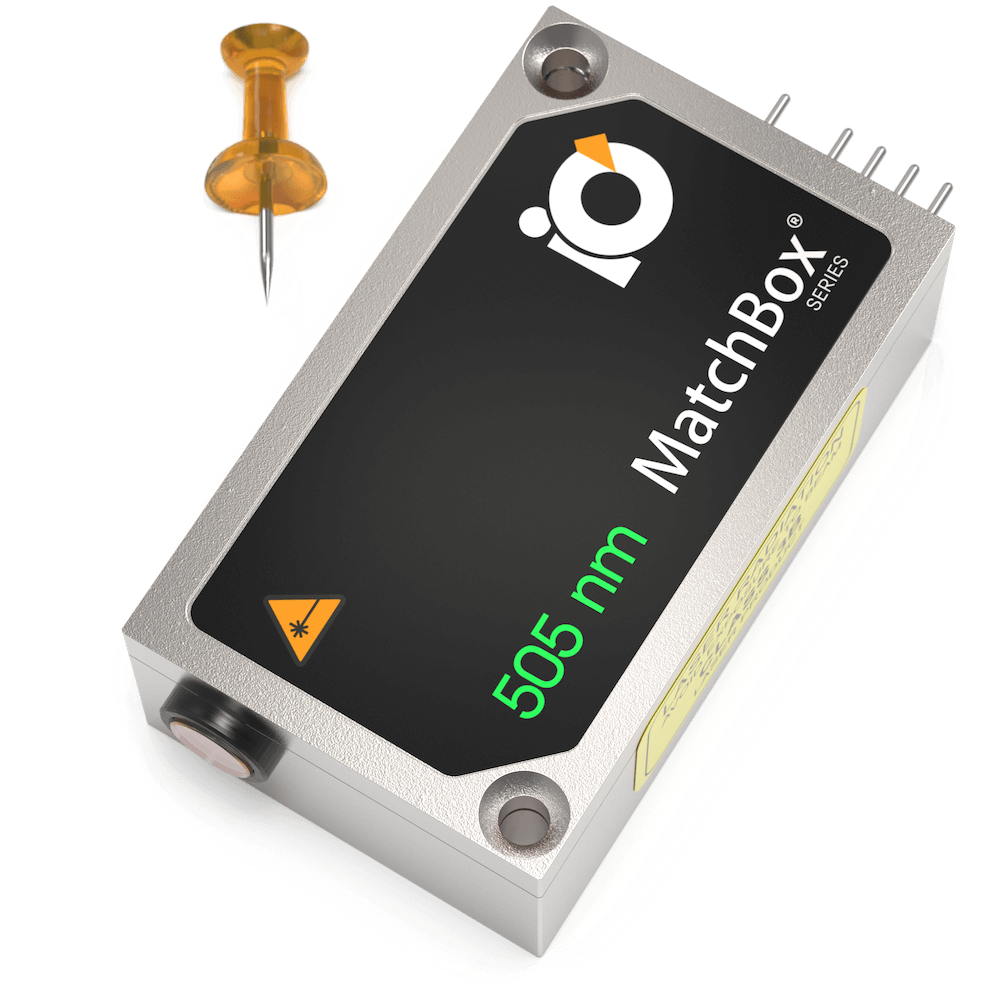
Multi-Wavelength Laser
488 nm - 40
520 nm - 80
638 nm - 130
Spectral line width FWHM, nm: 1
Power stability, % (RMS, 8 hrs): 0.2
Intensity noise, % (RMS, 20 Hz to 20 MHz): 0.5
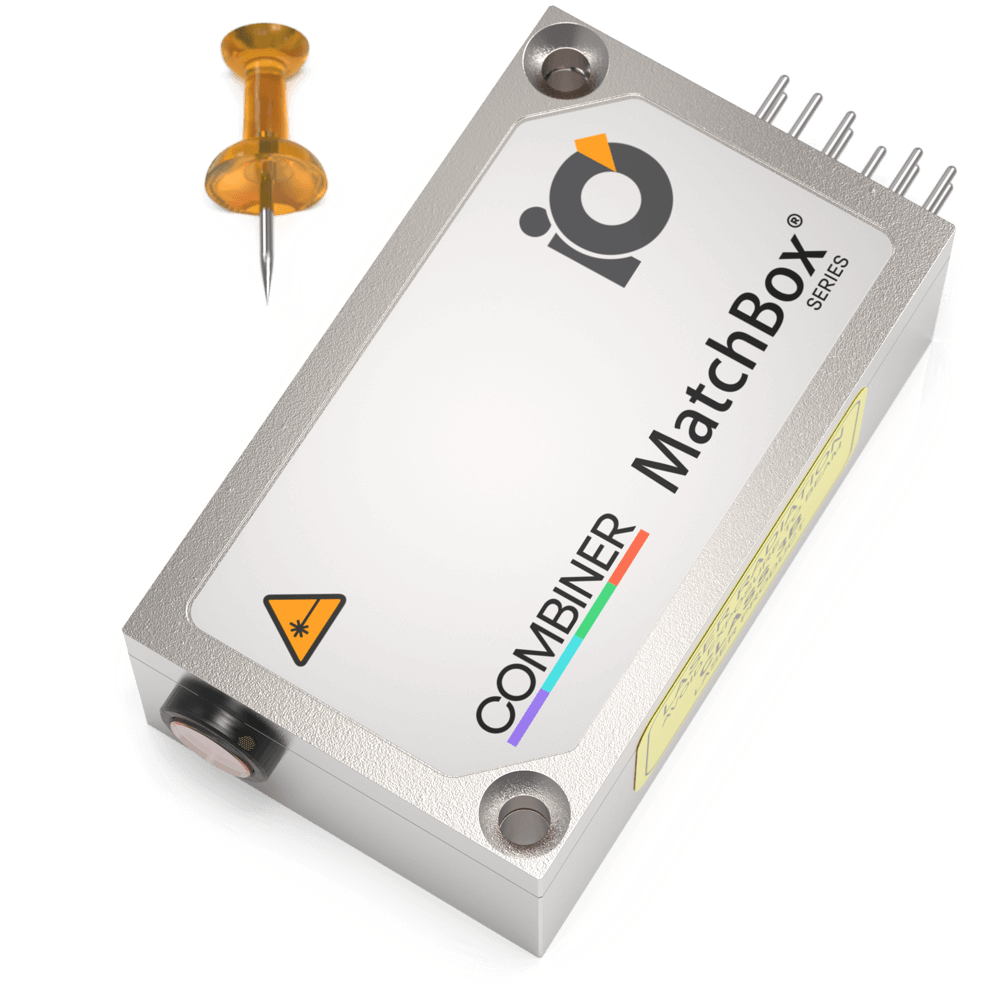
405 nm Laser
Spectral line width FWHM, nm: 0.5
Output power, mW: 180
Power stability, % (RMS, 8 hrs): 0.1
Intensity noise, % (RMS, 20 Hz to 20 MHz): 0.15
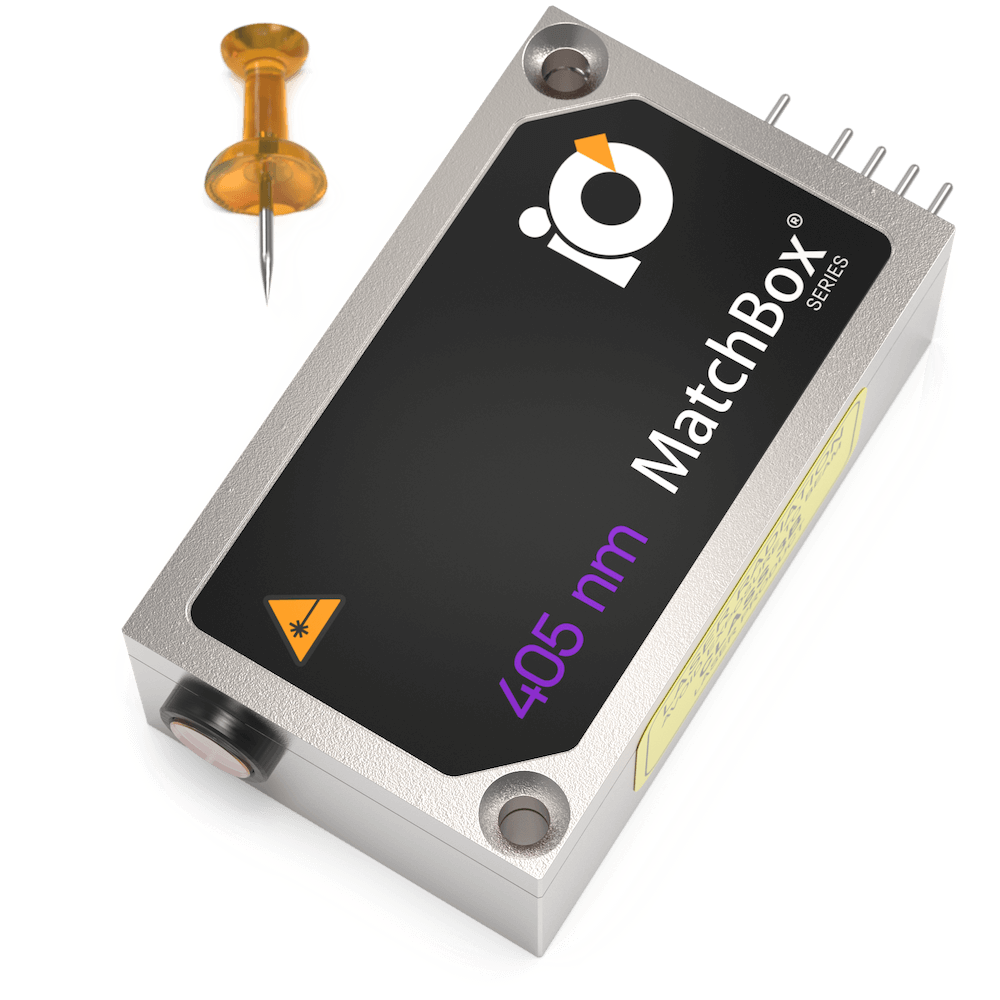
488 nm Laser
Spectral line width FWHM, nm: 0.5
Output power, mW: 40
Power stability, % (RMS, 8 hrs): 0.02
Intensity noise, % (RMS, 20 Hz to 20 MHz): 0.3
Warranty, months (op. hrs): 14 (10000)
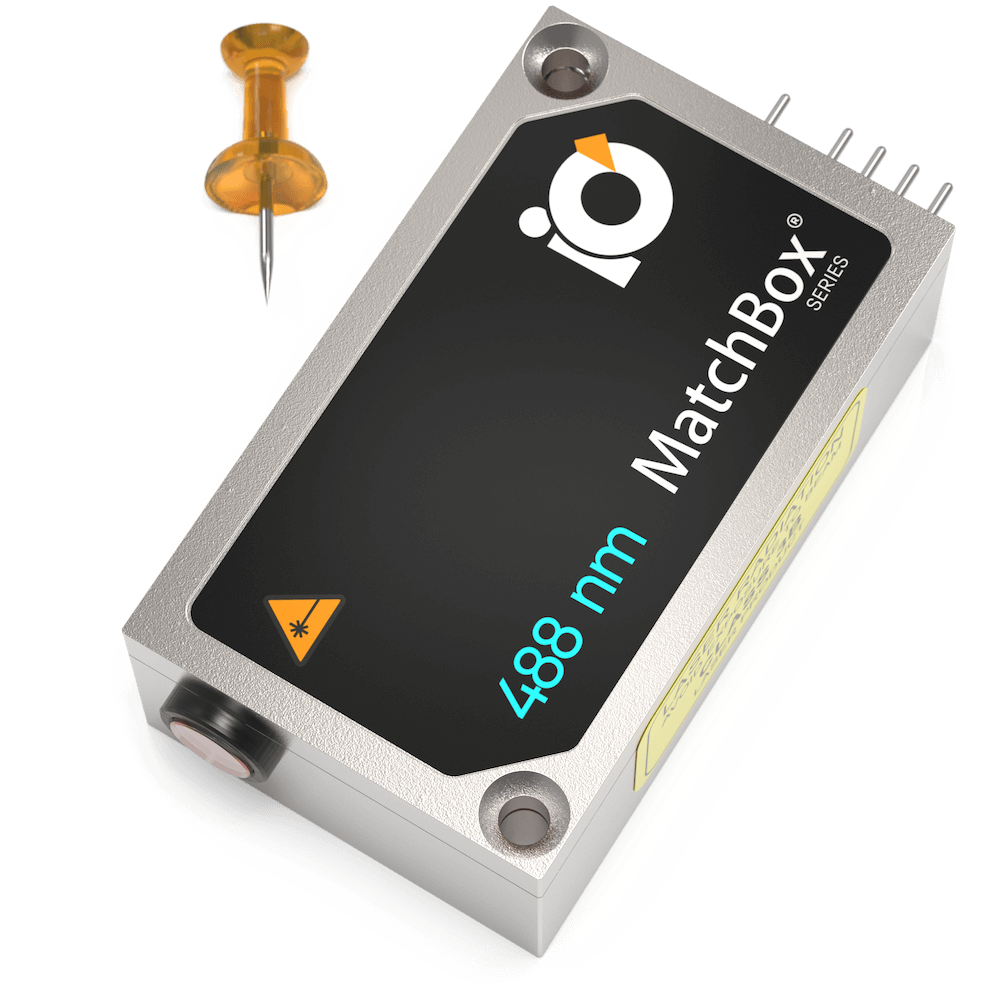